Date: March 6, 2023.
Imagine… A superhero universe where everything seems normal until one day, BAM! YOUR POWERS ACTIVATE and you’re DEADPOOL! Crazy regeneration power! Ringing any bells yet?
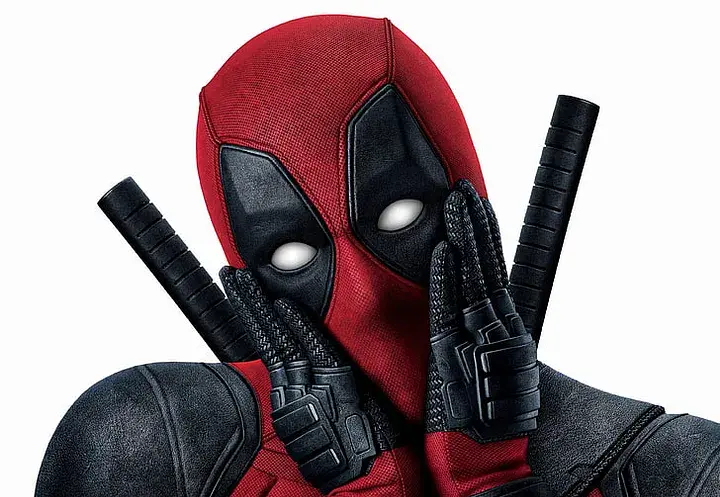
Well we are in the peak 21st century-4th industrial revolution-AI buzzing generation! And tons of action is happening in the lab! The superpower of pluripotency (ability of cells to become any cell in the body) can now be induced in mature adult cells!
Let us first dive into how we came into existence.
Your mother’s egg cell and your father’s sperm cell fuse and create a zygote (tiny single-celled you), then you start multiplying and create a ball, called a morula (you are 32 cells big now). Next, you convert into a blastocyst, from which emerge the superpower-holding stem cells. Now, in a process called gastrulation, your stem cells develop three germ layers.
Ectoderm (ecto- outside; derm- skin): Develops the epidermis (outer layer of skin), hair, nails, brain, spinal cord, and peripheral nervous system.
Mesoderm (meso- middle; derm- skin): Develops muscle, bone, connective tissue, kidney, gonads, and the circulatory system.
Endoderm (endo- inside; derm- skin): Develops the inner lining of the digestive tract; stomach, colon, liver, pancreas, bladder, and lungs.
Congratulations to you! In 10–12 weeks, with all your organs (except the brain and spinal cord, which continue to form and develop throughout pregnancy) formed, you have now become a foetus! At 20th week, your movements can be felt and finally at 40th week… you are born!
Happy Birthday to you!
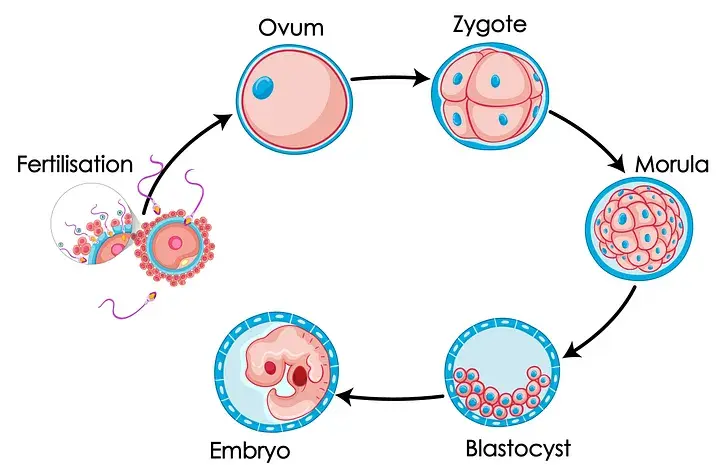
Based on different abilities, your stem cells are categorized as follows:
Totipotent: ability to differentiate into all cell types, capable of developing into a fully functional complete organism. Eg: a zygote cell.
Pluripotent: ability to differentiate into all three germ layers, ability to form any of the foetal or adult cell types. E.g.: embryonic stem cells.
Multipotent: lineage-restricted based on the organ of origin. E.g., hematopoietic stem cells only become blood cells.
Unipotent: ability to differentiate into a single type of cell. E.g.: epidermal cells produce skin.
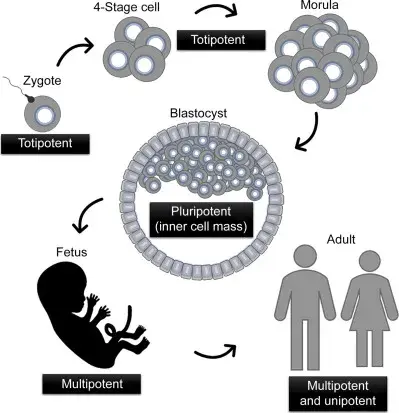
As you can see in this figure, your stem cells are only pluripotent while you are an embryo. However, as you transform into a foetus and later into an adult, they lose their pluripotency and become multipotent and unipotent. In other words, adult stem cells either possess limited differentiation potential or are capable of generating only a single specific cell type. The main function of these cells is to maintain the tissue’s homeostasis (the ability to maintain internal stability in response to environmental changes), that is, they can generate new cells that can replenish dead or damaged tissue.
Isn’t it fascinating how we went from a zygote to a fetus!
In 1950–1960, a young scientist named John Gurdon found out that the nucleus inside your cells has the machinery and genetic information needed to create different cell types, but it appears to turn off once the cell matures. This led him and other scientists to wonder-
What makes a stem cell a stem cell?
It wasn’t until later that researchers found out that a cocktail of factors were responsible for inducing pluripotency. By simply introducing these factors into a mature cell, one could manipulate them into believing and acting like a pluripotent stem cell.
It was now time to hunt for the right factors!
In 2006, the whole world’s paradigm shifted when Dr. Shinya Yamanaka reprogrammed somatic cells (the cells in the body other than sperm and egg cells) into a pluripotent state through the over-expression of several transcription factors (Yamanaka factors). These induced pluripotent stem cells (iPSCs) were largely identical with embryonic stem cells (ESC) and also demonstrated their ability to differentiate into derivatives of all three germ layers in vitro. The generation of iPSCs can be summarized in three major steps:
(i) Establishment of an initial cell culture.
(ii) The induction of iPSCs by the introduction of reprogramming factors (Oct3/4, Sox2, Klf4, and c-Myc).
(iii) Characterization and expansion of iPSCs.
There are two types of methods for the introduction of reprogramming factors:
Systems Integration/ Gene transduction — This approach includes fusing a fragment of a viral vector’s genetic material into the genome of the host cell. E.g. Retroviral or lentiviral. However, this could reactivate random exogenous (introduced/ produced outside the system) reprogramming factors and mess up the stability of iPSCs making it risky for insertional gene mutation and potential cancer transformation.
Non-integrated methods — They do not require the incorporation of transgenes (a gene or a segment of DNA that is introduced into the genome of an organism) into a donor’s somatic cell, thus eliminating the risks associated with integrated approaches and making it safer for potential clinical applications. E.g. Non-integrating viral vectors (sendai virus, adeno virus), plasmid DNA transfer, recombinant proteins, and synthetic mRNA. However, they have extremely low efficiency. Synthetic modified mRNAs have comparatively greater efficiency than other non- integrated approaches but RNAs have to be delivered into the cells daily during the reprogramming process.
These transfected cells are then incubated under appropriate media conditions and after the expression of these reprogramming factors, generation of iPSCs occurs.
This is a revolutionary breakthrough that is recognized all over the world! With just a tiny amount of somatic cells, we can induce pluripotency, expand the cells as much as we want, and ultimately create any tissues or organs we desire. It holds the potential to provide unprecedented opportunities for disease modelling, drug screening, tissue engineering and cell therapy.
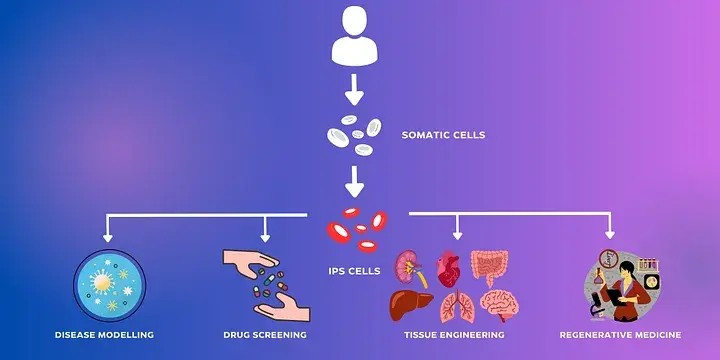
In 2007, a marked advance was made in the therapeutic use of iPSCs with the successful application of gene correction in a mouse model afflicted with sickle-cell anemia (a genetic blood disorder) — a landmark being set for regenerative medicine.
Compared with transplants from other donors, the iPSC-based autologous method is advantageous in that the risk of immunological rejection, transmission of unidentified viruses or other forms of infection is negligible. Animal models have contributed tremendously to a better understanding of disease mechanisms. However there are certain limitations when attempting to accurately replicate human diseases through animal models; illuminizing instances such as where drugs developed for rodent models of amyotrophic lateral sclerosis (ALS- a rare neurological disease that affects motor neurons and weakens muscles) proved inadequate on humans. Publications from two separate research teams in 2008 provided evidence for disease-specific, patient-specific iPSCs that enabled accurate reproduction of spinal muscular atrophy (SMA- a genetic disorder characterized by weakness and wasting (atrophy) in skeletal muscles). Great strides have been taken up until now creating several lines used for modelling rare illnesses and causing hope that these advancements will eventually lead to discoveries facilitating better treatment options.
Currently, many promising therapeutic applications are being tested throughout the world. CRISPR-Cas9 technologies are being used by stem cell biologists in order to repair mutated genes in human iPSC before using iPSC-derived cells to treat patients. They are being used in a variety of domains- Dermatology, Vascular therapy, Cardiology, Skeletal muscle regeneration, Neurology, Hearing loss, Ophthalmology, Bone and cartilage regeneration, Dentistry, Nephrology and urology, Pulmonology, Hepatology, Gastroenterology, and Metabolic disorders.
With such versatility and potential for therapeutic applications, iPSC technology holds a bright future towards a progressive future.
Author’s note: I have always been a curiosity bug. One day in secondary school, I asked my science teacher if regeneration was possible for humans; seeing my curiosity blossom into reality day by day gives me tremendous joy.
Comments